TWENTY-FIVE
They Thought the Sun Was Sick
After Ettore’s scomparsa, the neutrino—Ettore’s brainchild—went on to become a major player in particle physics. Throughout the 1950s it engendered respectable research, earning many a Nobel Prize. First neutrinos were actually “seen,” thanks to ghostbusters Reines and Cowan with their Project Poltergeist; no longer was the neutrino a mere theoretical expedient, as when Pauli first conceived it. Then the neutrino’s parity-violating properties were exposed by Lee, Yang, and Wu. The neutrino was chiral. Reality was stranger than fiction.
From then on, new facts about the neutrino regularly emerged. But calamities never come singly. Electrons and neutrinos belong to a family of particles dubbed leptons (particles that don’t partake in strong nuclear interactions). This family was about to expand.
Already in the 1930s, physicists examining cosmic rays (including Ettore’s five-minute friend Occhialini) had discovered a background of particles no one had anticipated. Among these some behaved just like the electron-positron duet in the reactions they caused: They had to be leptons. However, the new particles were much heavier than electrons or positrons: about two hundred times so. They were baptized “muons,” and it wasn’t until the 1940s (largely thanks to old Boy Bruno Pontecorvo) that scientists realized that the muon was partnered with a “muonneutrino.” In fact, the muon and its muonic neutrino were perfect replicas of the electron and the “electron-neutrino” as far as their reactions were concerned. In the same way that a neutron could decay into a proton, releasing an electron and a neutrino, heavy nuclear particles produced in cosmic-ray showers could decay by emitting a muon and a muonic-neutrino. Fermi’s weak force drove all these leptonic processes.
Later, in the age of giant particle accelerators, a third generation of leptons was exposed. In 1974, the group at SLAC accelerator, Stanford University, discovered the tauon, yet another facsimile of the electron, this time 3,500 times heavier. Having seen the pattern before, no one doubted that a tauon-neutrino should then also exist. But the tauon-neutrino wasn’t actually detected until 2000, at Fermilab. The complete lepton family had now arrived.
Electrons, muons, and tauons had their corresponding antiparticles: the positron, the antimuon, and the antitauon. As for their neutrinos, that depended crucially on their being Dirac or Majorana, and the issue remained open. Dirac neutrinos have distinct antiparticles; Ettore’s don’t. The fact that neutrinos were now known to come in three types didn’t change the outlook.
The neutrino has always lent itself to drama: You should therefore not find it totally unexpected that in this golden age of neutrino discoveries something catastrophic happened. It didn’t come from particle accelerators or nuclear physics labs, but from the sun. If you’re against nuclear energy, you should be campaigning against the sun: It is a nuclear device. And in the same way that nuclear reactors on Earth had been used to unveil the neutrino, the sun became a major asset for neutrino physicists. But something disastrous was found in the 1960s regarding the neutrino output of the sun.

The sun is nothing more than a natural nuclear power station, and as such it releases an abundance of neutrinos; indeed, the sun emits many more neutrinos than it does particles of light. Its nuclear reactions take place deep in the solar core, and the energy they release takes millions of years to reach the surface to be shed in the form of light. But because neutrinos are so tenuous and ghostly, they stream out of the sun in a matter of seconds, thereby offering us a real-time X-ray picture of the inner workings of the sun, ultimately allowing us to predict how its surface will shine in a million years.
In the late 1960s, John Bachall set out to compute the neutrino flux emanating from the sun, assuming basic nuclear physics and astrophysics. He then asked an experimental friend, Ray Davis, to detect these neutrinos and verify that it all checked out. It was a hard experiment because neutrino interactions are so rare. So to be certain the reactions he saw were not caused by mundane “ambient” radioactivity, Ray had to shield his apparatus very cautiously. He performed his experiments a few thousand feet underground in a mine in South Dakota. Only neutrinos can travel down that far; cosmic rays and other sources of interference are absorbed by the kilometers of rock above. Ray filled a container carried into these depths with six hundred tons of cleaning fluid, knowing that when a neutrino hits chlorine, the chlorine converts into radioactive argon gas, which he could measure.
John computed that Ray should see ten such events per week, all things considered (flux of solar neutrinos, probability of a conversion, and amount of chlorine.) Ray, instead, saw an average of around three: week after week, consistently, in clear defiance of John’s prediction.
When John and Ray hit the world with this surprising news, they were met with disbelief. If you can’t understand why, take a moment and consider the cheek of these two guys. One was 100 percent sure he knew how the sun worked and therefore how many neutrinos it emitted. The other claimed he could tell ten atoms apart within six hundred tons. And when he found three atoms instead, he was sure he hadn’t missed the other seven. Bachall and Davis had found a serious discrepancy between theory and experiment and wanted the world to address it. Not very surprisingly, they got a lot of hostile response instead.
At first, scientists blamed the experimentalist (always the easier scapegoat on such occasions): There had to be something wrong with Ray’s apparatus. The BBC Horizon programs of the 1970s make for some amusing viewing nowadays, when they featured reputable physicists lightly dismissing the whole thing, asserting that sooner or later the missing neutrinos would be found. But Ray Davis was sure of his experiment and stuck to his guns. He knew that if he had made a mistake and not properly shielded his device from ambient radioactivity, he’d be seeing an excess, not a deficit of neutrino events.
So then they blamed the theorist. Of course, John Bachall didn’t know what he was talking about—how could someone be so sure about the workings of the sun, such a complicated thing? Physicists thus played at ridiculing the theorist; the more redoubtable proposed alternative models of the sun, providing concrete new calculations of the neutrino flux. But the sun really isn’t that complicated and the variant models all gave the same neutrino flux, which seemed extraordinarily robust to details of solar structure. The only alternative solar models that produce fewer neutrinos were extreme and contrived, and were quickly ruled out by helioseismology—the study of solarquakes—an impressive method for obtaining information about the sun’s internal structure.
In between these two groups inescapably sat the alarmists—the wacky cults fond of eschatological prophecies. Perhaps the sun is shutting down. Maybe we were seeing a third of the expected neutrinos because only a third of its usual nuclear reactions were taking place at the solar core. The energy currently being released at its surface came from reactions that took place a million years ago. If only a third of these nuclear reactions were now taking place at the Sun’s core, then its energy output would decrease to a third over the next million years. Think of the dramatic implications on Earth! The end of the world is nigh.
The answer, however, was much simpler. The clue? The number three. The sun was emitting three times fewer neutrinos than it should. Not two, not five, but three.
The solution to the solar neutrino problem, as it happens, is vital to the controversy opposing Dirac and Majorana neutrinos—and the issue at its center concerns the neutrino mass.
But what is mass? The term has multiple meanings, both colloquially and in physics. Informally, mass means “amount of stuff” and weight is used to measure it. They’re conceptually different: Mass means “resistance to acceleration” or inertia, whereas weight is the ability to excite gravity. But to make a long story short, the two concepts—mass and weight—can indeed be used interchangeably (the long story being Einstein’s theory of general relativity).
Something else that can be confused with mass is energy, as you might have guessed from the famous equation E = mc2. According to this formula, energy—the ability to change the world—is equivalent to mass. The exchange rate is very large (the square of the speed of light), but again relativity conjures up the unification of seemingly different concepts. By adding energy to an object, you imperceptibly increase its mass: Something hot is “heavier” than something cold. And, likewise, mass itself constitutes a huge reservoir of energy, as nuclear physics and the world of particle annihilations spectacularly illustrate.
Weight, mass, and energy are thus equivalent within the framework of relativity. However, there’s one important distinction: “mass” versus “rest mass,” or “energy” versus “rest energy.” Motion is a form of energy,70 so E = mc2 implies that objects in motion are heavier or more massive than those at rest. In fact, that’s why you can’t accelerate an object to the speed of light: Its mass would become larger and larger, resisting the acceleration more and more. The mass of an object becomes infinite as it approaches the speed of light, and you simply can’t summon enough force to push it all the way up to the speed of light.
Rest mass is the mass (or energy) displayed by an object even when it’s at rest. The rest mass represents the object’s “internal” energy, which it preserves when it’s still, but which can be released by chemical or nuclear reactions. Particles with a rest mass can never move at the speed of light (they’d acquire infinite “moving mass” in the process). Conversely, particles that do move at the speed of light (like the photon) must have zero rest mass. A particle moving at the speed of light doesn’t have infinite mass because its rest mass is zero, or rather the particle can’t be seen at rest. Its energy derives from motion alone. Particles that move at the speed of light do so for all observers and are pure motion.
The world of particles is thus neatly divided between particles with zero and nonzero rest mass. Dropping the qualifier “rest,” particle physicists talk about massless and massive particles. The former move at the speed of light for all observers, the latter for none. The proton and the electron are massive, the photon is massless. How about the neutrino? According to the “standard model” of particle physics, developed in the 1960s, the neutrino is massless. But what if the standard model were wrong?

It’s historically curious that neither Pauli nor Ettore ever assumed the neutrino to be massless. If the neutron was proposed by Ettore as a “neutral proton,” required to bind the nucleus, the neutrino was envisaged as a “neutral electron” necessary to enforce energy conservation in beta decay. The neutron mass is only slightly different from the proton mass, but it was quickly realized that the neutrino mass had to be much smaller than the electron mass (for reasons to be made clear very shortly). Still no one assumed its rest mass to be zero.
Due to a number of mathematical problems, however, the new generation of neutrino physicists in the 1950s and 1960s posited that the neutrino moved at the speed of light and that its rest mass was zero. The only voice of dissent was Bruno Pontecorvo. He may not have been given a nocturnal induction exam by Ettore, but he surely came from the broadminded environment of Via Panisperna. There, no one had taken the neutrino to be massless.
But in 1967, Pontecorvo proposed something brutally insane, crazy beyond belief. At the time it was already established that neutrinos have “flavors.” This is a technical term in physics (not gourmet cuisine) referring to the three different generations of leptons discovered. The electron, muon, and tauon neutrinos can be seen as different “flavors” of the same particle. Pontecorvo knew that quantum mechanics is intrinsically odd—the Lord is malicious—and that sometimes different types of quantities we’re accustomed to measuring simultaneously become incompatible in quantum mechanics, ruled by mutually exclusive uncertainty. Position and velocity comprise one example, as I’ve explained before. If you know the exact velocity of a quantum car, you can’t know where it is (it must be in a quantum superposition of being at all locations). If you know its position, you have no idea of its speed. So position and velocity in quantum theory are “observables that don’t align”: They can’t be measured at the same time. Pontecorvo proposed that mass and flavor do not “align” for neutrinos.
This meant that if you knew the flavor of a neutrino, you couldn’t know its mass (you must be in a quantum superposition of different masses); if you knew its mass, you couldn’t know its flavor. Such a “mixing” of flavors and masses had a radical repercussion. If an electron neutrino is a superposition of different mass states (with well determined ratios), these masses will move at different rates through space. Thus the amount of the different masses will change as the neutrino progresses in space and time. And as the proportions change, so does the neutrino flavor: an originally “sweet” neutrino quickly turns “sour,” then “sweet” again—and so on, in a phenomenon called “flavor oscillation.” An electron neutrino oscillates into a muon and tauon neutrino as it moves, due to its funny kind of mass (see Figure 25.1). The rate at which the oscillations take place depends on the difference between the various mass states and something Pontecorvo dubbed the mixing angle. For typical values, the oscillations complete a cycle every few kilometers.
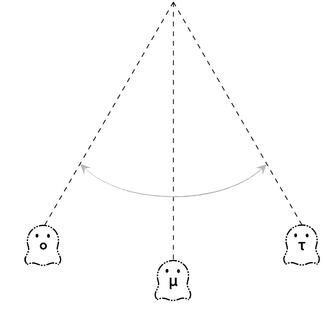
Pontecorvo oscillations. As the neutrino moves in space, it oscillates between being an electron neutrino, a muonic neutrino, a tauon neutrino, then back again.
For a while, physicists continued to play the blame-the-experimentalist, blame-the-theorist, blame-the-sun game regarding the solar neutrino puzzle. But in the 1980s and 1990s, a Japanese group studying neutrinos produced by cosmic rays in the atmosphere found a similar discrepancy. They were also seeing fewer neutrinos than they should. The particle created by Pauli to account for missing energy was itself showing a knack for going missing. And when a larger Japanese experiment confirmed yet again a generalized neutrino deficit, physicists realized the game was over. It was time to start blaming the particle itself for the anomalies.
It was at this point that Pontecorvo’s idea—largely disregarded up until then—began to gain respect. Pontecorvo had suggested that not only did the neutrino have a mass, but also that its mass states didn’t align with its flavor states. As a result, an electron neutrino oscillated into a muon neutrino, which oscillated into a tauon neutrino, and finally back to an electron neutrino to restart the cycle. Ray Davis’s solar neutrino experiment was only sensitive to electron neutrinos. No one had objected because the sun can produce only electron neutrinos in its nuclear reactions. But what if neutrinos oscillated? Two-thirds of the emitted electron neutrinos would on average be lost to muon and tauon flavors as they moved toward us. This would solve the solar neutrino problem. It was just a matter of putting three and three together. Three generations or flavors; three times fewer neutrinos seen (see Figure 25.2).
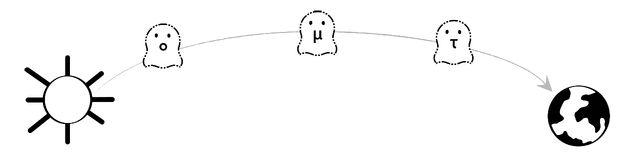
Figure 25.2: The (electron) neutrinos produced by the sun oscillate en route to Earth. Since the oscillation period is a few kilometers, and they came from a much broader region at the solar core, at any place on Earth we see on average a third of the total solar neutrino output in each flavor: electron, muon or tauon.
It was a disarmingly simple solution to the solar neutrino conundrum, but there was only one way to verify that it was indeed the correct answer: to devise an experiment that could detect all three types of neutrinos. SNO—Solar neutrino observatory—started running in 1999, three kilometers (almost two miles) underground in a mine at Sudbury, Canada. It’s now the cleanest environment in terms of ambient radioactivity in the whole solar system. You need to shower and decontaminate yourself thoroughly before they’ll let you anywhere near. It was in such an exclusive location that physicists waited for ticks in their detectors, signaling the catch of another neutrino of any flavor coming from the direction of the sun.
The answer provided by SNO was unambiguous: Taking into account all types of neutrinos, the sun was in fine health, thank you very much. John Bachall’s calculation was correct: The flux of solar neutrinos was as predicted. And Ray Davis was measuring things right, too. He just wasn’t seeing all the flavors that issue from the sun because he had restricted himself to electron neutrinos. The sun creates only electron neutrinos in its reactions, but it just so happens that Pontecorvo’s wild guess hit the spot. Neutrinos have mass but their mass states don’t align with their flavors, and so the flavors oscillate.
As a result, a third of the solar neutrinos arrive on Earth as muon-neutrinos, and another third as tauon-neutrinos. There are more things in heaven and earth, Horatio, than are dreamed of in your philosophy. And this one couldn’t be more relevant for the outcome of Ettore’s story.