TWO
Nuclear Crisis
Just to make things more theatrical, Ettore’s science itself revolved around energy gone missing. Had he worked on time machines, conspiracy theorists couldn’t have had more fuel. But it’s a fact: For most of Ettore’s life, no one knew where vast amounts of energy had gone. Energy was seemingly disappearing from the face of the Earth, unaccounted for, evaporated forever. Perhaps this might not sound so bad in Sicily, where “people have a knack for vanishing,” as I was once told at a café in Catania. Skeptical, I picked up the local paper, La Sicilia. To the amusement of my interlocutor, right there on the front page was the “storia triste” of a certain Rita Cigno, “la donna scomparsa,” who’d vanished into thin air twelve years before. The family had enlisted the services of a clairvoyant who had just been jailed for fraud. I recalled Signora Nunni Cirino telling me how she’d been pestered by a similar professional of the occult.
Whether it’s OK or not for people to vanish (not always to be found dead at the bottom of a pit), the very Sicilian Ettore was associated with an energy sink on a cosmological scale. But rather than add to this mystery, he helped solve it. Only to leave us with a larger conundrum, deep in the waters near Catania, as that other newspaper clipping—the one that prompted me to board a plane to Sicily and write this book—had hinted. Deep in those waters lies Ettore’s Nobel Prize.
You already know what it’s for: the neutron’s sissy little brother, the neutrino.

E = mc2: Energy has mass, and mass is worth energy. That’s what the most famous formula in the world tells us. The exchange rate is very large, the square of the speed of light, c2, but conceptually matter and energy are equivalent: two sides of the same coin. Neither can be created or destroyed; they can only metamorphose, change in form and type, the overall amount of mass-energy always remaining the same. “In nature nothing is lost, nothing is gained, everything transforms,” as in the adage of Antoine Lavoisier. When I launch a rocket into space, quite a lot of energy in the form of motion is gained, but it’s not created out of nothing: It is born out of the chemical energy spent in burning fuel. When two particles collide and new ones are created, the type of mass-energy changes, but not its total amount. Conservation of mass-energy is one of the most sacrosanct principles of modern science, and it’s as if Ettore’s disappearance was the final twist in a long drama entailing energy destruction.
Yet that’s precisely where Ettore’s main discovery lies. It concerns “vanishing energy” and how it revealed a new particle, pathologically shy, almost impossible to detect: the neutrino. A perfect analogy between discoverer and discovered, observer and the observed, Ettore and his neutrino—an analogy spoiled only by the neutrino’s lack of a domineering mother.
Instead, the neutrino had a domineering father: the often revered, always feared Wolfgang Pauli.
A brilliant Austrian physicist, “Pauli’s mere presence in a city [was] enough to make all experiments go wrong,” it was universally said. In the vicinity of theoretical physicists, his effect was similar: To him everything was ganz falsch, “utterly false,” and he could be awfully nasty in his delivery of criticism. He was highly respected because his opinions were often, if not always, correct and also because he had equally high standards with regard to his own ideas. When he met the Dutch physicist Paul Ehrenfest, the latter felt compelled to remark, “I think I like your papers better than you.” To which Pauli replied, “I think I like you better than your papers.” The two became good friends.
A well-known anecdote describes Pauli, after his death, being granted an audience with God to discuss the secrets of the universe. After watching the old man scribble a profusion of formulae on the blackboard, Pauli explodes: “Das is ganz falsch!” Even God spoke out of His ass, according to Pauli.
But Pauli was also a complex man who drank way too much and endured long periods of extreme depression. His mother had committed suicide, and his father got remarried to a woman Pauli loathed, traumatizing him for the rest of his life. By 1930, Pauli had gone through several unhappy relationships, including a very short-lived marriage. (His wife dumped him for a chemist: “Such an average chemist,” he commented.) In 1931, he had a severe nervous breakdown and became a long-term correspondent and friend of the psychoanalyst Carl Jung, who treated his “neurosis.” Ettore was nicknamed Il Grande Inquisitore; Pauli was sometimes labeled the “conscience of physics.” It seems that such roles come with a price.
In a historic letter dated December 4, 1930, Pauli wrote to the famous nuclear physicist Lise Meitner—“Dear Radioactive Ladies and Gentlemen”—and proceeded to apologize for his intended absence from an important meeting due to take place in Tübingen, Germany. He explained that “a ball which takes place in Zürich on the night of the 6th to 7th of December makes my presence here indispensable.” The meeting in question was intended as a forum to discuss an extraordinarily grave problem in nuclear physics, something that had triggered a major crisis, casting doubt upon the respectability of the whole field of research. Pauli had sorted out his priorities correctly, favoring dance and flirtation over saving science from the perils of self-contradiction; but as a consolation in his letter to Meitner he offered a solution to the puzzle. “I dare not publish anything about this idea,” he wrote, before branding his solution “a desperate way out.”
In his letter, Pauli proposed a new particle—the neutrino, which he’d later call “that foolish child of the crisis of my life.” A few months before, he’d divorced his wife after less than a year of married life; shortly afterwards, Jung had to record four hundred of his dreams before he was deemed “treated.” Perhaps the ball he went to in Zürich wasn’t wild enough; perhaps giving birth to an idiosyncratic new particle wasn’t particularly fulfilling for him. As a memento of his frustrations, the particle has stayed with us.

With the massive benefit of hindsight—and simplifying a situation that was far, far more complex—I could shamelessly summarize nuclear physics in the early 1930s as follows: We’re made of atoms, which have a nucleus with a positive charge surrounded by clouds of orbiting electrons with a negative charge (see Figures 2.1 and 2.2). The chemical properties of a substance result from the actions of the outer electrons and their ability to bring atoms together into molecules. Explaining the behavior of these electrons is the subject of atomic physics, but by the 1930s a new branch of physics had emerged concerned with the nucleus: nuclear physics. This was to open up the world to a whole new “chemistry” no one had yet sampled.
Figure 2.1: The three main characters in the universe. The proton and the electron have the same charge but with opposite signs (positive for the proton, negative for the electron.) The neutron is electrically neutral. The caricatures’ mouths are meant as mnemonics.
Figure 2.2: An atom as we understand it today (not to scale, and with a very large pinch of salt). The nucleus is made up of protons and neutrons. Electrons whizz around the nucleus at high speed.
Figure 2.3: An illustration of the numbers A and Z labeling any element and isotope, where we chose lithium (A = 7 and Z = 3) as an example. The lithium nucleus is inhabited by three protons and four neutrons.
The nucleus was known to contain two types of particles: protons, with a positive charge, and neutrons, electrically neutral (see Figure 2.1). Each type of nucleus, associated with each element in the periodic table, was characterized by two numbers: A and Z. A referred to the total number of protons and neutrons; Z to the total number of protons. The total number of neutrons was therefore A minus Z. For example, gold has A = 197 and Z = 79; that is, its nucleus has 79 protons and 118 neutrons. Lithium (used in many laptop batteries) has A = 7 and Z = 3 (see Figure 2.3).
In the early 1930s, we knew the world was made of the elements in the periodic table, but not exclusively: Radioactive isotopes were regularly being discovered. A given element in the periodic table has fixed A and Z values, but trace amounts of “twins” of these elements had been discovered in nature, with the same Z but a different A. For example, most oxygen atoms in nature have eight protons and eight neutrons, but 0.2 percent of oxygen atoms have eight protons and ten neutrons.
Such nonidentical twins were called isotopes. Because they had the same Z, and thus the same outer electrons responsible for chemistry, they had the same chemical reactions and other similar properties.11 In an isotope, only the nucleus was different, because it contained a different number of neutrons. The overall mass per atom was therefore different, but other than that, chemistry was blind to the distinction between an element and its isotopes (see Figure 2.4).
Figure 2.4: The three isotopes of hydrogen (Z = 1). Regular hydrogen (with A = 1) has only one proton in its nucleus. Deuterium (A = 2) has a proton and a neutron. Tritium (A = 3) has a proton and two neutrons. The nuclei of all these isotopes are orbited by a single electron (not represented) and so have the same chemical properties.
These alternative versions of the elements existed in tiny amounts in nature, and it was difficult to “distill” or isolate them. But they had a striking property: Most of them naturally disintegrated into other elements or isotopes and in the process gave off especially energetic radiations. Because this phenomenon was first discovered in the element radium, it was called radioactivity. The process had astonishing features.
The energy associated with the radiations given off by radioactive disintegration was much, much higher than that in chemical processes: about a million times higher! Thus, those who studied nuclear physics must have realized at once that they had in their hands an enormous energy source. Explosives, at the time, resulted from particularly energetic chemical reactions. I wonder who first made this alarming connection.
Three types of radioactive reactions were known, associated with the three types of radiation that they gave off. They were labeled alpha, beta, and gamma. Alpha radiation was the least energetic and could easily be stopped with a sheet of paper. An alpha particle was made up of two neutrons and two protons, and it signaled the transmutation of a given element into another element with A reduced by four and Z reduced by two (see Figure 2.5).
Beta radiation, on the contrary, signaled a “sex change” in a neutron within the nucleus. Somehow a neutron became a proton, emitting very energetic radiation that could be stopped only by sheets of aluminum. Later, scientists understood that the stability of the nucleus is the result of balancing the number of neutrons and protons (something Ettore helped explain, as we shall see). If an isotope had a surplus of neutrons with respect to the optimal number, it was liable to convert one of its neutrons into a proton, leading to a transmutation into an element with Z increased by one but with the same A value (see Figure 2.6). In the process, it emitted the very energetic beta radiation, soon identified with an electron moving close to the speed of light. Electrons emitted during beta radiation are not to be confused with those orbiting the nucleus, as they are emitted directly by the nucleus and are much more energetic.
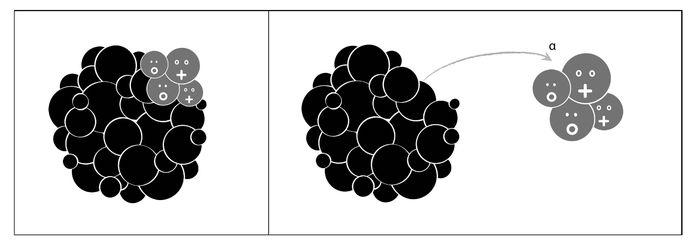
Figure 2.5: In alpha decay, a nucleus ejects an alpha particle (constituted by two protons and two neutrons), thereby losing two of its protons and two of its neutrons. After alpha decay, the nucleus transmutes into another with A reduced by four and Z reduced by two.
Figure 2.6: In beta decay, a neutron inside the nucleus converts into a proton, emitting a very energetic electron: the beta ray. Thus the daughter nucleus has the same A as the mother, but its Z is increased by one.
By far the most powerful form of radiation was gamma radiation. This was an extraordinarily energetic particle of light, a photon (see Figure 2.7). No transmutation occurred as its result; rather, gamma radiation was emitted when the nucleus shook off surplus energy after one of the other types of radioactive disintegration had taken place. Gamma rays could be stopped only by very thick layers of lead and were by far the most biologically destructive of the radioactive radiations. Mutations, cancer, and tissue damage were among the horrors gamma rays could inflict (see Figure 2.8).
Figure 2.7: A gamma ray is a particle of very energetic light. It signals an energy offload by the nucleus, typically after undergoing one of the other types of decay. In gamma decay, the nucleus is simply letting off steam, and its A and Z remain unchanged.
Figure 2.8: Alpha particles can easily be stopped by a sheet of paper. For beta rays, a sheet of aluminum might be a safer bet; whereas for gamma radiation, a thick layer of lead is the only protection against severe radiation damage.
It was quickly understood that radioactivity was precisely the ancient dream of alchemists! It opened up the doors for a new type of “chemistry,” in which elements transmuted into each other, for the number of protons and neutrons in nuclei did change during a radioactive process. By contrast, in chemical reactions, atoms merely changed their organization into molecules, or collections of atoms, but the nature of each individual atom remained unchanged. Turning sulfur into gold required nuclear physics. It was the “chemistry” of radioactivity that finally realized the original but failed motivation for chemistry—the philosopher’s stone: the key to alchemical transmutation into gold.

What I’ve just described in a couple of pages originally took over thirty years to clarify. And although it’s an oversimplification, with appropriate poetic license I can justifiably say that by the early 1930s, nuclear physics had pretty much fallen into place except for one tricky detail: the spectrum of beta decay. It was no laughing matter, and one must sympathize with the man who’d rather go to a ball than rack his brains about it. But, as usual, the problem was solved by the dancing grasshopper, while the ants labored at the lab. The matter that concerned all those ants, due to gather in Tübingen while Wolfgang Pauli licked his emotional wounds in Zürich, was simple: Energy was disappearing.
One can portray Ettore’s 1938 eclipse as vanished energy, but that’s only a metaphor. What was happening during beta decay was, by contrast, brutally concrete. A mother nucleus transmuted into a lighter daughter nucleus, and if you multiply their difference in mass by the square of the speed of light, according to the dependable E = mc2, you obtain the amount of energy that should be carried away by each electron making up beta radiation. But the electron invariably had less energy. Much less.12
Figure 2.9: The spectrum of beta radiation. By considering the mass difference between mother and daughter nucleus, and using E = mc2, one finds the “available” energy which should be carried by the beta ray. But it invariably carries less energy.
Where the hell was this energy going (see Figure 2.9)?
The energy, quite possibly, was going nowhere. It was just disappearing, and our principle of conservation of energy was wrong. Such was the opinion of Niels Bohr, who in a lecture given in May 1930 stated, point blank, “At the present stage of [nuclear] theory we may say that we have no argument, empirical or theoretical, for upholding the energy principle in the case of beta-ray disintegrations, and are even led to complications and difficulties in trying to do so.” He was well aware of what he was insinuating—he added, “Of course a radical departure from this principle would imply strange consequences.” However, he was prepared to take the leap and conclude, “[Still, the facts] may force us to renounce the very idea of energy balance.”
I like Bohr’s courage, but his was really a lazy explanation. After all, the same violations of energy conservation didn’t plague the other radioactive processes, alpha and gamma emissions, and Bohr offered no explanation for this. This logical flaw excited Pauli’s acerbic criticism; and thus in his December 1930 letter to Lise Meitner, Pauli suggested the presence of “a third man” to solve the mystery (see Figure 2.10). Of course energy was conserved—Bohr was a total donkey. It was just that an invisible third particle was taking it away. The neutrino was born.
Figure 2.10: The full picture of beta decay according to Pauli. A ghost particle, the neutrino, carries away the missing energy.
What Pauli did is a common form of scientific witchcraft: He willed something into being by the power of logic and generalization. It’s called making a prediction, and it is quite similar to what happens when a jealous wife finds that her husband is spending nights away from home. She postulates the existence of a lover even though she hasn’t actually seen one. And, of course, in due time she’s usually proved right.
For example, in the 1840s, French astronomer Urbain Le Verrier predicted the existence of the planet Neptune to explain strange perturbations in the orbit of Uranus. He claimed that this “Neptune” planet was too far away from us and therefore too faint to have been observed thus far, but that it must be there because its pull on Uranus was detected. He even directed astronomers as to where and when to look for the new planet. And when they pointed their telescopes to the appropriate spot they duly found the ghost planet, within a degree of Le Verrier’s pronouncement. It was in this tradition that Pauli proposed the existence of the elusive neutrino. As Pauli put it, “I admit that my expedient may seem rather improbable from the first. . . . Nevertheless nothing ventured, nothing gained. . . . We should therefore be seriously discussing every path to salvation.”
But after so much boldness, Pauli remained cautious about his creation. Admittedly he was suffering from a nervous breakdown, for unrelated reasons. But after his famous letter to Lise Meitner, excusing himself from the meeting and proposing the neutrino, the next time he aired his brainchild was on July 31, 1931, almost eight months later. Grossly overweight and in pain from a broken shoulder (having fallen down some stairs while drunk), Pauli finally owned up to his creation at a lecture in Pasadena, California. Even then he refused to write up his lecture; but a journalist attended it, and the New York Times reported on the neutrino hypothesis. For once, the overly keen science reporter was correctly ahead of the scientist.
We should not be too tough on Pauli, however. The luminaries of the time dubbed his idea “implausible,” “crazy,” or “simply wrong,” and for a good reason. Some time later, Rudolph Peierls computed that so intangible was the neutrino that we’d need a few light-years of lead to stop it and make it interact with our detectors. That’s a few million times the distance between the sun and Earth—in lead. So, the neutrino was not a ghost, but it was close.
Perhaps a bit like Ettore as a child.